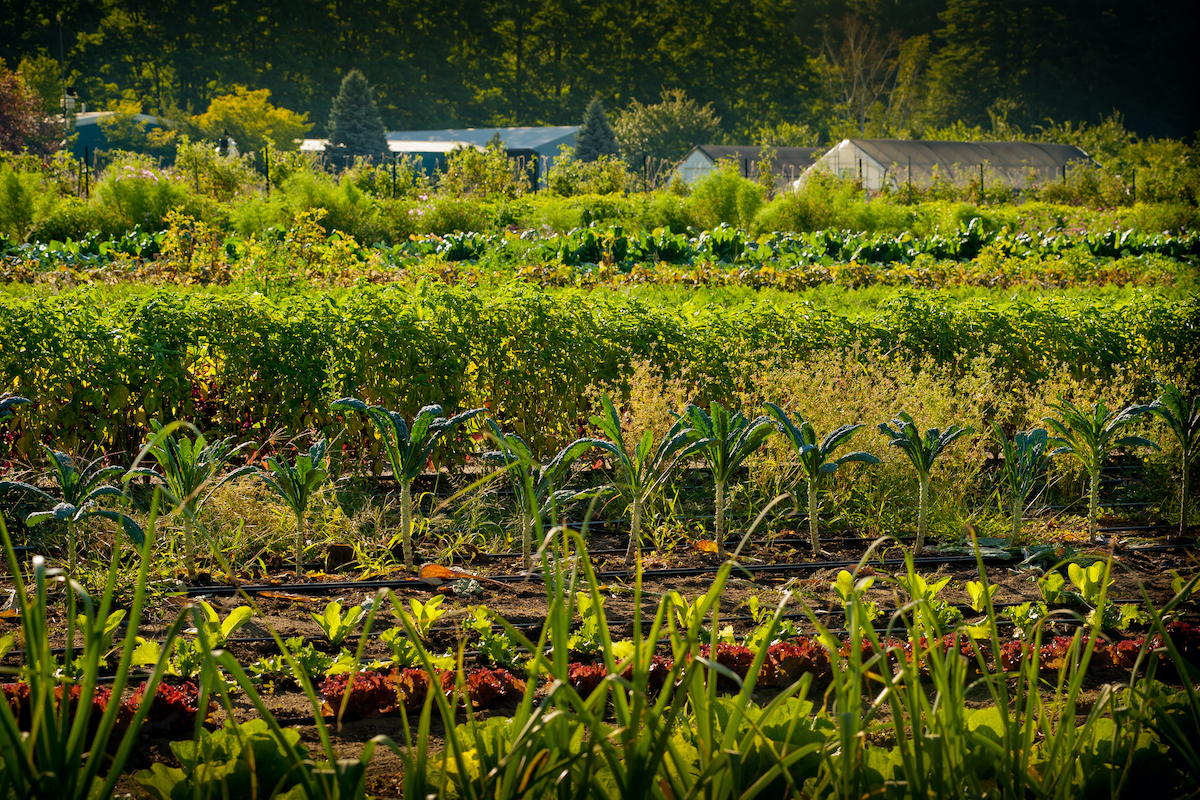
Carbon Sequestration in Agricultural Soils
Download chapter PDF and figures
Authors
Eric D. Roy
Rubenstein School of Environment & Natural Resources, Department of Civil & Environmental Engineering, and Gund Institute for Environment, University of Vermont, Burlington, VT 05405
Catherine E. Horner
Department of Plant & Soil Science, and Gund Institute for Environment, University of Vermont, Burlington, VT 05405
Citation
Horner, C., Roy, E., 2021. Carbon Sequestration in Agricultural Soils. In Galford, G.L., Faulkner, J. et al. (Eds), The Vermont Climate Assessment 2021. Burlington, Vermont: Gund Institute for Environment at the University of Vermont. DOI: 10.18125/kowgvg.
Table of Contents
- 10.1 Key Messages
- 10.2 Summary Points
- 10.3 Introduction
- 10.4 The Biogeochemistry of Climate Mitigation by Agricultural Soils
- 10.5 The Available Evidence Linking Practices to Climate Mitigation
- 10.6 Monitoring and Considerations for Payment Schemes
- 10.7 Recommendations for Next Steps in Vermont
- 10.8 Traceable Accounts
- 10.9 References
10.1 Key Messages
- The soil carbon sequestration potential of agricultural management practices in Vermont is uncertain and likely mediated by site-specific factors such as soil type, geography, land use history, and weather. Climate change mitigation benefits are possible but not guaranteed from the use of common practices implemented to sequester carbon (such as cover cropping, conservation tillage, no-till, and rotational grazing) on Vermont agricultural lands. There is evidence, however, that these practices can improve soil health and increase farm resilience to climate change.
- Assigning carbon offsets or payments for climate mitigation services provided by Vermont agricultural lands based on practice adoption alone currently lacks a strong scientific foundation. Further investigation and monitoring is needed to improve understanding of management practices and soil carbon sequestration, including field studies and modeling. Well-calibrated models, validated for application in Vermont, have potential for identifying relationships between management change(s) and carbon dynamics. Participatory research that engages the expertise and needs of farmers is necessary to assess the local impacts of best management practices and make projections into the future.
- Whole-system accounting is required to assess potential trade-offs and to determine net climate change mitigation benefits of soil management strategies. Changes in soil carbon stocks at a given location are only one piece in climate mitigation accounting. In all cases where offsite carbon sources are being used to boost soil organic carbon, a broader life cycle assessment extending beyond the farm gate is needed that considers offsite carbon source removal, transport, and processing; alternative end uses of the carbon source; interactions with other soil GHG-producing processes; and synergies between the soil amendments and the input of in situ plant-derived carbon. It is critical to keep in mind the primary objective: increase the net transfer of CO2-equivalents from atmosphere to land. Only strategies achieving this primary objective should be considered climate mitigation. Failing to account for other fluxes of carbon and greenhouse gases could result in unintended consequences due to trade-offs.
10.2 Additional Summary Points
- It is imperative to ground statewide climate mitigation efforts in Vermont firmly within the most recent scientific evidence and to highlight key scientific uncertainties and knowledge gaps that have policy implications.
- The soil carbon sequestration potential of agricultural management practices is inconsistent across the literature and is mediated by site-specific factors such as soil type, geography, land use history, and weather.
- More research is needed to improve understanding of how management practices affect both shallow (0-30 cm) and deeper (>30 cm) soil layers.
- Climate change mitigation benefits are possible but not guaranteed from the use of common practices implemented to sequester carbon (such as cover cropping, conservation tillage, no-till, and rotational grazing) on Vermont agricultural lands. However, there is consistent evidence that these practices improve soil health and increase farm resilience to climate change.
- Monitoring is necessary to quantify climate mitigation benefits of specific practices applied across multiple Vermont agricultural contexts.
- Well-calibrated models, validated using local data, hold promising potential to identify relationships between management change(s) in a particular context and ensuing change(s) in net C fluxes, but such models do not yet exist for application in Vermont.
- Assigning carbon offsets or payments for climate mitigation services provided by Vermont agricultural lands based on practice adoption alone currently lacks a reliable and consistent scientific foundation.
- Changes in soil carbon stocks are only one piece in climate mitigation accounting. Whole-system accounting is required to assess potential trade-offs and to determine net climate change mitigation benefits of soil management strategies.
- Farm resilience is vital, and farmers should be supported in implementing best management practices for improving financial and ecological resilience.
- Participatory research that engages the expertise and needs of farmers is necessary to assess the local impacts of best management practices and make projections into the future.
10.3 Introduction
Agricultural soil health has become an increasingly popular topic in Vermont and other New England states in the past 10 years. From scholarship (Jemison et al., 2019; Kersbergen, 2012; Bakelaar et al., 2016; Adair et al., 2019) to popular news coverage (Foster, 2021; Leslie, 2020), the topic is receiving considerable attention. Amidst the growing interest in soil health, there is a particular focus on the potential for agricultural soils to sequester atmospheric carbon and thereby contribute to the mitigation of climate change. In the State of Vermont’s 2021 legislative session, bills were proposed to: establish a statewide definition of and commitment to soil health and implement carbon sequestration tax credits as part of a statewide payment for ecosystem services program. Interest in soil carbon was also evident in a 2020 survey administered as part of the UVM-ARS partnership. Survey respondents identified soil organic matter and active carbon as two of the five most important metrics for assessing soil health on Vermont’s small- and medium-sized farms. Similarly, respondents identified interactions between soil health and climate as one of the top three research and outreach priorities for the University of Vermont (Neher et al., 2021).
Interest in agricultural soil carbon sequestration in Vermont is likely influenced by large-scale policies and initiatives established elsewhere. This includes the “4 per 1000 Initiative” for increasing soil organic carbon stocks, also known as “4 per mille” or “4‰,” which was launched by the French Ministry of Agriculture in 2015 for the 21st Conference of Parties of the United Nations Framework Convention on Climate Change (UNFCCC). This initiative aspires to increase global soil organic carbon stocks by 0.4% per year to offset the global emissions of greenhouse gases (GHGs) by anthropogenic sources. The initiative mainly focuses on agricultural soils with relatively low levels of soil organic carbon and encourages farm management practices that preserve and increase soil organic carbon stocks while limiting carbon trade-offs (Rumpel et al., 2020). While the goals of climate change mitigation and increasing soil organic carbon are certainly laudable, the 4 per 1000 Initiative has received significant criticism from several scientists (Poulton et al., 2018; Rumpel et al., 2020). The potential for “regenerative agriculture,” which has been defined in various ways (Newton et al., 2020), to sequester carbon in soils and thereby serve as a climate mitigation tool has also been the focus of intense scientific debate. For example, the World Resources Institute recently posted a blog entitled “Regenerative Agriculture: Good for Soil Health, but Limited Potential to Mitigate Climate Change” (Ranganathan et al., 2020), which engendered both support (Powlson et al., 2020) and criticism (Paustian et al., 2020) from well-respected scientists. While these debates are particularly intense at the moment, they are not entirely new (e.g., Schlesinger, 2000).
It is imperative to ground climate mitigation efforts in Vermont firmly within the most recent scientific evidence and to highlight key scientific uncertainties and knowledge gaps that have policy implications. In this chapter, we first briefly describe the underlying biogeochemistry relevant to climate mitigation by agricultural soils. Second, we review evidence linking practices associated with “conservation agriculture” or “regenerative agriculture” with climate mitigation outcomes (carbon emissions, soil carbon storage, nitrogen emissions). Third, we discuss challenges related to measurement and potential payment schemes. Finally, we close with recommendations for future efforts in Vermont.
10.4 The Biogeochemistry of Climate Mitigation by Agricultural Soils
Soils’ physical, chemical, and biological properties and processes are interconnected and can all be considered aspects of “soil health” (Moebius-Clune, 2016). This is especially important in the context of climate mitigation, for which the cycling of carbon and nitrogen is key. Biogeochemistry is a systems science related to the field of ecosystem ecology that integrates physics, chemistry, biology, and geology (Schlesinger and Bernhardt, 2013), providing a powerful lens for examining potential climate mitigation by agricultural soils. Using this lens, some key facts relevant to the assessment of climate mitigation by agricultural soils are:
- Changes in soil organic carbon storage are driven by the balance between carbon inputs to and losses from the soil. Carbon inputs to soils can include plant litter (shoots and roots), root exudates, and exogenous soil amendments (e.g., manure, compost, or biochar) (Basile-Doelsch et al., 2020). Carbon loss from soils mainly occurs as CO2 resulting from autotrophic (roots) and heterotrophic (microorganisms and fauna) respiration, but can also include CH4 emissions that result from methanogenesis under anaerobic conditions, leaching of dissolved organic carbon, and erosion (Basile-Doelsch et al., 2020).
- There are two primary levers to increase soil organic carbon storage – increase C inputs and/or decrease C losses. Increases in carbon inputs to agricultural soils can potentially be driven by improved crop rotations and increased crop residues, use of cover crops, conversion to perennial grasses or legumes, additions of manure, compost, or biochar, or improved grazing land management (Paustian et al., 2019). Decreases in carbon losses can potentially result from conversion to perennial grasses or legumes, using conservation or no tillage, or rewetting soils (Paustian et al., 2019).
- Soil organic carbon storage does not necessarily increase linearly with increased C inputs – and there are limits. While some long-term agricultural field studies have observed soil C stocks that appear linearly related to the amount of C returned to the system, others show little or no change in soil organic carbon in response to varying C input levels, or decreased soil organic carbon stabilization efficiency in high carbon soils compared to low carbon soils for the same treatment (Stewart et al., 2007). Saturation limits to soil organic carbon pools have been proposed or observed by various researchers with emphasis on certain mechanisms (Hassink, 1997; Baldock & Skjemstad, 2000; Stewart et al., 2007; Owen et al., 2015; Poulton et al., 2018). It may be possible to shift a soil’s effective C stabilization capacity to some degree through changes in soil management (Stewart et al., 2007) and more research is needed to better understand the related mechanisms that determine limits on soil organic carbon storage (Basile-Doelsch et al., 2020). Despite this uncertainty, it is generally agreed that the greatest potential for soil carbon sequestration exists on degraded soils with relatively depleted soil organic carbon (Lal, 2018; Amelung et al., 2020).
- The cycling of other elements, particularly nitrogen and phosphorus, affects soil carbon storage and greenhouse gas emissions. Nitrogen addition stimulates plant growth when N availability is a limiting factor and thereby increases C inputs to the soil (Huang et al., 2020). The same is true for phosphorus. These two elements (and others) must be available in the soil in adequate amounts to maintain crop productivity and resultant C inputs to the soil, which has led some to caution that ambitious global goals for carbon sequestration in agricultural soils will require vast inputs of N and P to soils (Van Groenigen et al., 2017; Spohn, 2020). Furthermore, nitrogen can be lost from agricultural soils as nitrous oxide (N2O), an important greenhouse gas, which must be considered along with soil carbon storage and carbon emissions (CO2, CH4) in the determination of net climate mitigation benefits (Owen et al., 2015; Guenet et al., 2020).
10.5 The Available Evidence Linking Practices to Climate Mitigation
10.5.1 Croplands
The Nature Conservancy has recently created AgEvidence, a web-based tool to explore the impact of agricultural practices on crops and the environment (http://www.agevidence.org/, doi:10.5063/Z31X15). AgEvidence compiles evidence from numerous peer-reviewed field studies conducted in the US Midwest between 1980 and 2020 that focus on the links between agricultural practices and in-field response variables. All field trials included in AgEvidence focused on corn (including sweet corn), soybean, or both. For climate mitigation, AgEvidence includes studies examining the effects of cover crops and tillage. Figure 10-1 below summarizes 277 observations from twenty-seven studies on climate mitigation effects of cover cropping, including carbon emissions, carbon storage, and nitrogen emissions.
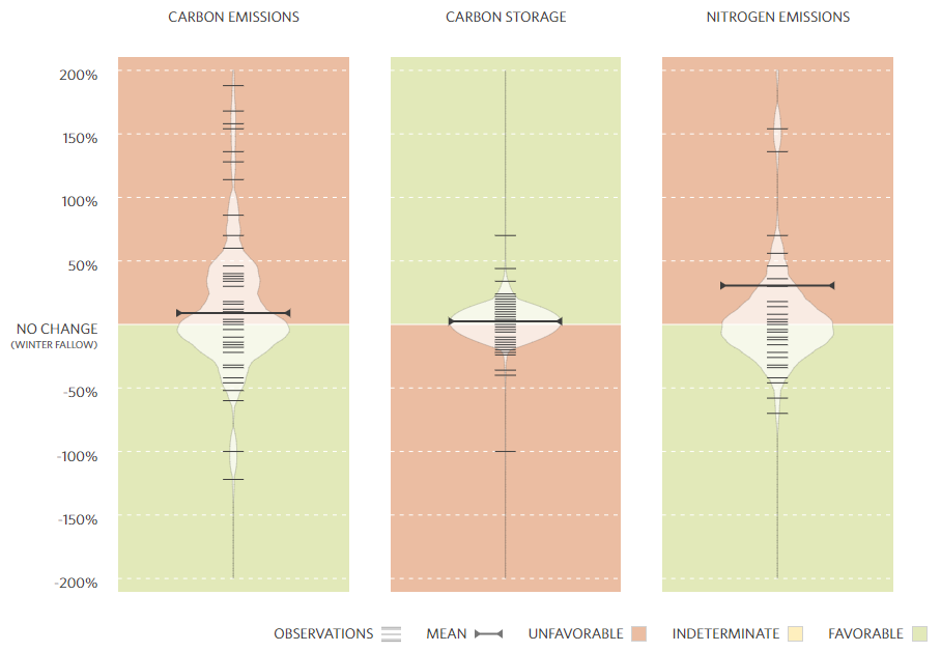
A companion “Insight” box on the AgEvidence website states that “cover crop studies show some potential for climate mitigation” and summarizes the evidence for cover crops and climate mitigation as follows:
- The use of single species, non-leguminous cover crops is associated with a 3% increase relative to the control for soil carbon on average. (Note: there are far fewer observations associated with multiple species cover cropping systems in AgEvidence).
- Soil carbon increases linked to cover crops are greater in topsoil (0-30 cm), whereas when the 0-60 cm depth was analyzed, changes in soil carbon linked to cover crops were neutral to slightly negative.
- Caution is warranted when interpreting nitrogen and carbon emissions in Figure 10-1. Further inspection of the data (see Figure 10-2 below) shows that cover crop usage is associated with no change in year-round N2O emissions, while growing season measurements show an increase in N2O with cover crops largely due to results from a single study where irrigation was present, which is known to increase N2O emissions. Offseason N2O emissions are also slightly increased with cover crops; however, this result was strongly influenced by a single study from a manure impacted soil. The study with irrigation also drives the pattern of higher CH4 emissions with cover crops.
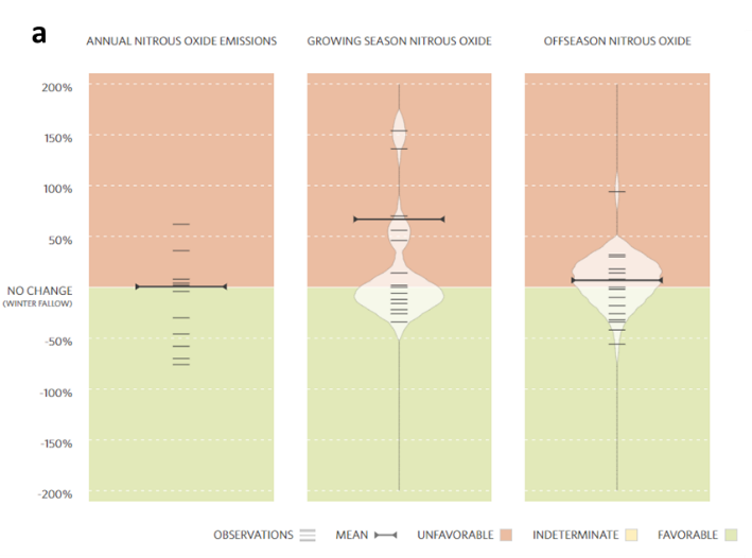
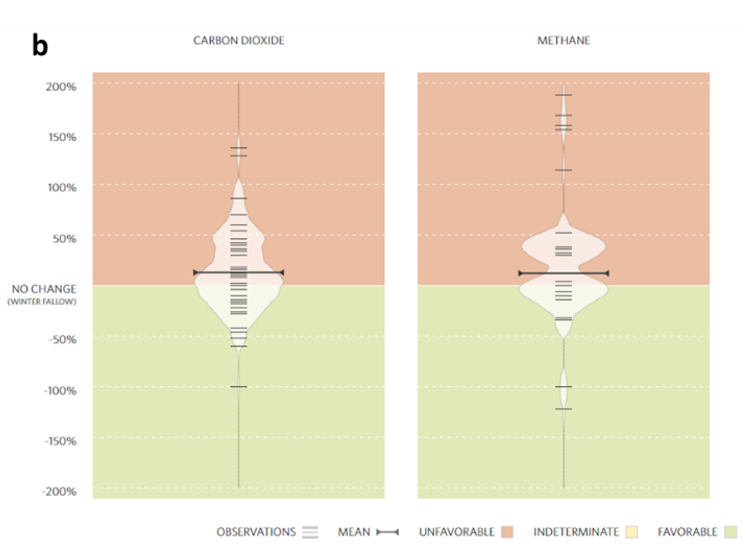
Some recently published global meta-analyses provide favorable results for the effect of cover crops on soil carbon storage. Poeplau and Don (2015) found that cover crop treatments had significantly higher soil organic carbon stocks compared to reference croplands, with an annual increase of 0.32 ± 0.08 Mg C ha-1 yr-1. These findings have recently been used as the basis for large-scale estimates of climate mitigation by cover crops in Canada (Drever et al., 2021). However, the mean soil depth of sampling in the studies considered by Poeplau and Don (2015) was only 22 cm, indicating that their assessment could be optimistic given the less promising results in AgEvidence when deeper soils were included. Furthermore, many of the studies considered by Poeplau and Don were short-term (2-3 years), which is generally an insufficient time duration to assess changes in stable forms of soil organic carbon (Smith, 2004). Jian et al. (2020) found that including cover crops in rotations significantly increased soil organic carbon in near-surface soils by 15.5% and investigate the influence of climatic region, soil texture, cover crop types, cash crop types, and soil sampling depths. For example, they report that soil organic carbon increases were greatest for fine-textured soils. However, Jian et al.’s (2020) meta-analysis results were also largely influenced by short-term (< 5 years) studies of surface soils (0-30 cm), which suggests their conclusions could also be optimistic. More research is needed to clearly demonstrate the long-term effects of cover crops on soil carbon storage generally and within Vermont specifically.
Figure 10-3 below summarizes observations of tillage effects on climate mitigation, including carbon emissions, carbon storage, and nitrogen emissions. Effects of conservation tillage (Figure 10-3a) and no-till (Figure 10-3b) are shown separately. As with cover crops, a wide range in effects of conservation tillage or no tillage spanning from unfavorable to favorable has been reported in the peer-reviewed literature, with generally favorable mean changes relative to conventional tillage.
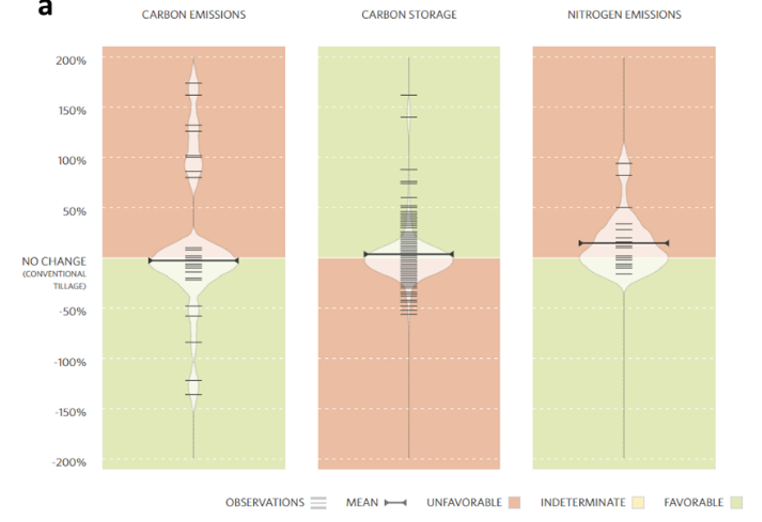
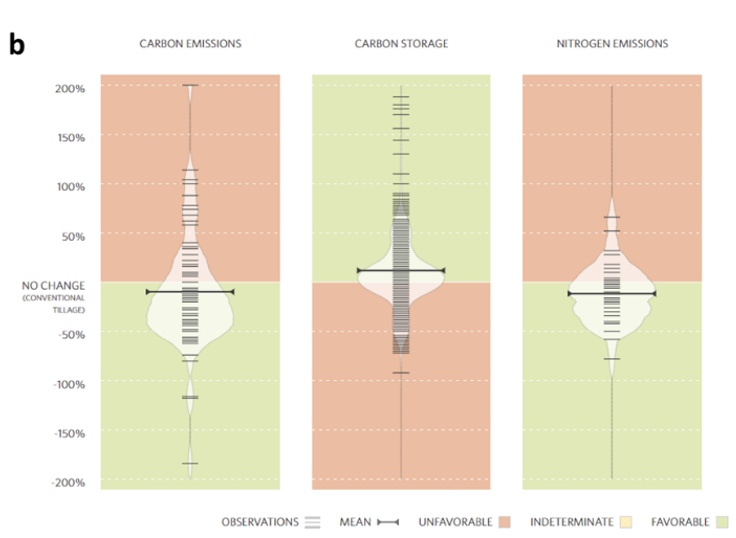
Based on the AgEvidence database, conservation tillage results in mean percent changes relative to conventional tillage of -2.6% (favorable) for carbon emissions, +3.7% (favorable) for carbon storage, and +14.7% (unfavorable) for nitrogen emissions. For comparison, no-till results in mean changes relative to conventional tillage of -9.7% (favorable) for carbon emissions, +11.8% (favorable) for carbon storage, and -11.7% (favorable) for nitrogen emissions. These results suggest that the benefits of no-till for climate mitigation are on average greater than those associated with conservation tillage. An “Insight” box on the AgEvidence website states, “No-till is associated with greater carbon storage, lower methane emissions, and lower growing season N2O emissions” compared to conventional tillage. However, other researchers report evidence that in some cases increased N2O emissions in no-till systems can offset the increased soil C storage when both fluxes are compared in CO2-equivalents (Guenet et al., 2020).
One important methodological factor to consider in studies of no-till and soil carbon sequestration is the depth of soil considered in the analysis. Previous researchers have noted that observations of increased carbon storage based solely on measurements in the 0-30 cm soil layer can be misleading (Baker, 2007). This is due to the fact that crop roots often extend much deeper than 30 cm and increases in soil organic carbon (SOC) within surface soils may be accompanied by losses of SOC at greater depths (Baker, 2007; Luo et al., 2010; Powlson et al., 2014; Olson and Al-Kaisi, 2015; Slessarev et al., 2021). AgEvidence also lends some support to this concern, with the caveat that fewer studies have examined deeper soil layers (Figure 10-4). For the 41 studies in AgEvidence focused on the 0-30 cm soil layer, a mean change in soil carbon of +15.5% (favorable) for no-till relative to conventional tillage was observed (Figure 10-4a). However, the mean change in soil carbon for the 16 studies that examined 0-60 cm, 0-100 cm, or 0-150 cm soil layers drops to -0.3% (neutral/unfavorable) for no-till relative to conventional tillage (Figure 10-4b). Therefore, more research is needed to enhance understanding regarding how changes in tillage practice affect both shallow (0-30 cm) and deeper (>30 cm) soil layers.
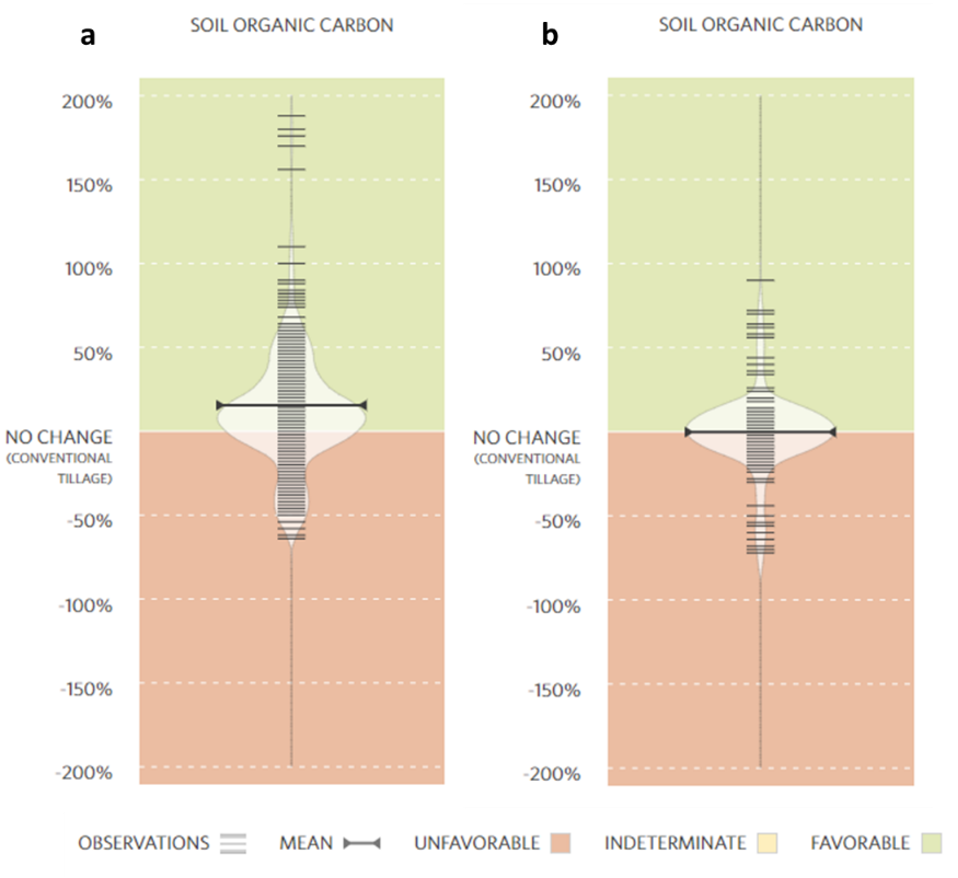
The data summarized by AgEvidence provide a useful starting point for assessing the potential climate mitigation effects of management practices on croplands in Vermont. However, AgEvidence includes no data from Vermont and it is important to ask how the differences between Vermont and the Midwest, in terms of climate, soils, and other factors, might affect the climate change mitigation potential of various management practices. Unfortunately, we do not have enough local data to answer that question at this time. A search of peer-reviewed literature using Web-of-Science (terms: Vermont* soil* carbon*) on May 9, 2021, returned only one local agricultural field study. Dittmer et al. (2020) tested the effects of different management practices on GHG emissions in Alburgh, Vermont, over three growing seasons and found that: (a) no-till reduced CO2 emissions but had no impact on N2O emissions relative to vertical till, and (b) manure injection increased N2O and CO2 emissions, with the magnitude of this effect being greatest for 1 month post-application. We recommend that additional Vermont data be collected and systematically assessed in the future.
In summary, the evidence reviewed here collectively indicates that climate mitigation benefits are possible but not guaranteed from the use of common conservation practices (cover cropping, conservation tillage, no-till) on Vermont croplands. Inconsistency in the effects of practices on climate mitigation outcomes across studies could be influenced by numerous site-specific factors (time duration, weather, soils, other environmental factors, land management, and/or sampling methodology). The paucity of peer-reviewed Vermont evidence further increases uncertainty in the potential for climate mitigation from conservation practices on croplands in the state. Therefore, in the opinion of the authors, assigning carbon offsets or payments for climate mitigation services provided by croplands based on practice adoption alone in Vermont currently lacks a scientific foundation and should therefore be avoided unless new evidence becomes available. Monitoring is necessary to verify climate mitigation benefits. More discussion of monitoring is presented below.
Another potential strategy to boost soil organic carbon on Vermont croplands is the input of manure, compost, or biochar. It is clearly possible to build soil organic carbon in a field through the addition of manure or other organic amendments, which contain carbon themselves as well as nutrients that can stimulate plant growth resulting in conversion of atmospheric CO2 into plant biomass C (e.g., Liang and MacKenzie, 1992; Poulton et al., 2018). However, taking a broader view beyond a single field, this practice does not necessarily result in a net sink for C in soils (i.e., additional transfer of carbon from atmosphere to soil) (Schlesinger, 2000). Adding more manure or compost to one field typically means adding less to another, thereby representing a reallocation of C between terrestrial locations. Biochar shows some promise for enhancing soil carbon sequestration in agriculture; however, biochar supply, cost, and uncertainty or time lags for climate mitigation benefits remain hurdles both locally and globally (Woolf et al., 2010; Shackley et al., 2014; Smith, 2016; Campbell et al., 2018). In all cases where off-site C sources are being used to boost soil organic carbon, a broader life cycle assessment extending beyond the farm gate is needed that considers off-site C source removal, transport, and processing; alternative end uses of the C source; interactions with other soil GHG-producing processes; and synergies between the soil amendments and the input of in situ plant-derived C (Paustian et al., 2016). It is critical to keep in mind the primary objective: increase the net transfer of CO2-equivalents from atmosphere to land – only strategies achieving this primary objective should be considered climate mitigation.
10.5.2 Grazing Lands
Grazing strategies, especially rotational grazing, are receiving increasing national and global interest as potential tools to sequester soil organic carbon and enhance soil health more broadly (Derner et al., 2016; Schulz et al., 2016), with ongoing debate centered on the potential benefits of grazing management to improve agricultural production and ecological outcomes (Roche et al., 2015). Rotational grazing strategies include, for example, high-intensity short duration grazing, “mob” grazing, and management-intensive grazing (Byrnes et al., 2018). Byrnes et al. (2018) conducted a global meta-analysis including 64 research articles to examine the effects of grazing strategy (i.e., no grazing, continuous grazing, rotational grazing), grazing intensity (light, moderate, heavy grazing), and site-specific environmental factors on three important aspects of soil health: soil carbon, nitrogen, and bulk density. Their results can be summarized as follows (Byrnes et al., 2018):
- Increased soil compaction occurs under all grazing strategies and intensities relative to no grazing.
- Rotational grazing strategies improved soil organic carbon and bulk density conditions over continuous grazing strategies.
- Greater continuous grazing intensity levels can negatively affect soil organic carbon.
- Site specific environmental factors underpin soil function and health responses to management.
Others have found that stocking rate, coupled with effective livestock distribution, is the single most important management variable influencing production and conservation goals in grazed ecosystems, outweighing other aspects of grazing strategy (Briske et al., 2011).
More research is needed to evaluate the climate mitigation potential of regenerative grazing systems, especially considering the multiple grazing approaches currently being explored by producers (e.g., holistic, adaptive, and other variants of rotational grazing). One challenge with such research is that adaptive grazing systems, by definition, are dynamic in response to varying weather and other environmental conditions that affect grassland productivity. It is therefore difficult to set up traditional replicated field experiments to compare different grazing systems at the landscape scale (Paustian et al., 2019; Teague et al., 2013).
Some recent evaluations find that climate mitigation estimates for regenerative grazing systems have been exaggerated (e.g., Nordborg, 2016), and others suggest some short-term promising results (Stanley et al., 2018; Mosier et al., 2021). A recent study by Rowntree et al. (2020) examined the ecosystem impacts and productive capacity of a multi-species pastured rotation (MSPR) livestock system at the well-known regenerative White Oak Pastures farm in Georgia, and report results that highlight some key points:
- First, implementation of the MSPR system on degraded cropland resulted in substantial soil carbon sequestration (a 5-fold increase in SOC stocks over 20 years) (Rowntree et al., 2020). While this is impressive, soil carbon gains following conversion of degraded cropland to pasture is not necessarily surprising given what is known about relative carbon storage across ecosystem types (Amelung et al., 2020). Furthermore, others have argued that, from a global perspective, opportunity to convert annual cropland to grazing is limited by the growing need for annual crops, noting that even if diet changes or yield gains could free up cropland, that land could sequester more carbon if restored to forest (Searchinger and Ranganathan, 2020).
- Second, when incorporating soil carbon sequestration, life cycle assessment indicated that the MSPR system emitted 4.1 kg CO2-equivalent per kg of carcass weight, 66% lower than the comparative conventional commodity system (Rowntree et al., 2020). Although this constitutes a substantial reduction, the net GHG footprint of this MSPR system is still positive, drawing into question previous claims that the MSPR system has a ‘carbon negative footprint’ (White Oaks Pastures, 2019).
- Third, the MSPR system required 2.5 times more land compared to a conventional commodity system to produce the same amount of meat on a per carcass weight basis (Rowntree et al., 2020). The authors point out that the MSPR is well-suited for marginal lands, rather than productive lands suitable for higher value and more nutrient dense crops (Rowntree et al., 2020). However, it is again important to note the fact that such marginal lands could also be suitable for restoration to non-agricultural ecosystems that provide numerous ecological benefits (forests, wetlands). Agroforestry systems could be explored as well.
In a recent article entitled “Soil carbon sequestration in grazing systems: managing expectations,” Godde et al. (2020) summarizes the state of knowledge on grazing systems and climate mitigation as follows:
- Grazing systems emit greenhouse gases, which can be partly or entirely offset by soil carbon sequestration under specific agro-ecological conditions.
- Any soil carbon sequestration in grazing systems is time-limited and reversible.
- Protecting large carbon stocks in grazing lands is essential in order to avoid further CO2 release.
- Soil carbon sequestration in grazing lands should be promoted in cases where it delivers environmental and agronomic benefits, including on degraded land.
- Caution should be applied for estimates of climate mitigation by C sequestration in grazing systems due to large uncertainties and dependence on economics, feasibility of implementation, and time frame considered.
10.6 Monitoring and Considerations for Payment Schemes
Despite the fact that measurement of soil carbon stocks in a single soil core sample is relatively straightforward, there are considerable challenges to accurately estimating changes in soil organic carbon stocks at field and farm scales. These include high spatial variability, changes in bulk density with changes in soil management, potential for redistribution of soil organic carbon between surface soils and deeper soils, and the slow rate of changes in bulk soil carbon (Smith et al., 2020; Slessarev et al., 2021). If feasible, soil organic carbon monitoring should include analysis of soils to at least 60 cm depth, and ideally to 100 cm depth (Liebig et al., 2010; Slessarev et al., 2021). However, measuring below 30 cm (the minimum recommended depth by IPCC; Ogle et al., 2019) can increase cost and require specialized equipment. It is not currently possible to verify sequestration rates that increase soil organic carbon stocks by <1% on an annual basis using direct soil measurements (Paustian et al., 2016; Smith et al., 2020). Therefore, longer study periods (ideally > 5 years) that extend beyond the timeline of most research grants are required to track changes in soil organic carbon (Smith et al., 2004). Measurement of total organic carbon by dry combustion is recommended, with steps taken to subtract any inorganic C present (Liebig et al., 2010; Nelson and Sommers, 1996). Researchers also commonly estimate soil organic matter using the loss on ignition (LOI) method (Nelson and Sommers, 1996). However, the relationship between LOI and soil organic carbon varies across soils and conversions between LOI and soil organic carbon should be validated using location- or soil-specific data (Konen et al., 2002).
There are some promising spectral methods emerging for direct point measurements in the field and lab (Smith et al., 2020). These include methods for measuring SOC concentration based on the reflectance of light on soil in the infrared region, where the organic bonds and minerals in the soil absorb light at specific wavelengths and SOC is predicted using a statistical model based on a spectral library (Smith et al., 2020 and citations within). In addition, laboratory costs could be reduced by using Fourier transform mid-infrared diffuse reflectance spectroscopy for estimation of total carbon, organic carbon, clay content, and sand fraction (Wijewardane et al., 2018). Remote sensing methods could also potentially be employed at scales not feasible with traditional or point spectral methods (Ge et al., 2011; Mulder et al., 2011). However, there are limitations of remote sensing, including limited penetration depth, limited duration of bare soil, and cloud cover (Smith et al., 2020). Furthermore, soil bulk density is a critical measure when estimating soil organic carbon stocks and cannot be estimated using remote sensing. Some techniques have been developed for estimating bulk density using gamma-ray attenuation and visible-near infrared spectroscopy on cores in the field to reduce costs and errors associated with transport, handling, oven-drying, and laboratory measurements (Lobsey and Rossel, 2016). Considering field study results discussed above, any approach taken will ideally need to be capable of characterizing bulk density in deeper soils (> 30 cm in depth) for tracking soil carbon stock changes. Combining traditional field/lab methods (including sample compositing schemes to reduce cost) with emerging spectral methods could help expand the areal extent of monitoring feasible at reasonable cost and should be explored further (Smith et al., 2020; Slessarev et al., 2021).
Another approach that can be employed in the tracking of soil organic carbon is modeling. Many models exist that estimate soil organic carbon dynamics; however, calibration and validation of such models is difficult and not all relevant biogeochemical processes are represented (Smith et al., 2020 and citations within). Some existing modeling tools, such as the Cool Farm tool and the COMET-Farm tool, have existing platforms that can be used to estimate net GHG emissions changes based on farm practices (Cool Farm Alliance, 2019; NREL, 2019). We recommend that the use of models be explored in Vermont as a means of increasing the spatial extent for which soil organic carbon accounting can take place, with one essential caveat: models must be properly calibrated using local data to establish confidence in the model results provided. This inevitably requires field and lab measurements to establish acceptable statistical relationships between modeled and measured values from (a) local benchmark sites undergoing long-term experiments to inform model parameterization, and (b) well-characterized chronosequences or paired sampling sites (Smith et al., 2020). It could be prohibitively expensive to set up benchmark sites covering all possible combinations of land use, climate, soil type, and management practice, so models will likely need to be tested across the full range of parameter space to allow reliable simulation of soil organic carbon (Smith et al., 2020). Without such grounding, models remain unverified abstractions of reality based on untested assumptions, and therefore should not be trusted. This becomes especially concerning in a situation where financial payments or carbon offsets are being determined for climate mitigation services. Well-calibrated models, supported by local measurements, could eventually be used to establish relationships between a management change in a particular context (soil, climate, land use, management) and a change in net C fluxes, including estimates of uncertainty, allowing management data reported by farmers to become adequate for reporting and reduce the need for direct measurements (Fitton et al., 2017; Smith et al., 2020). In the views of the authors, this is not yet currently possible in Vermont.
Additionally, whole systems accounting is needed in order to assess potential trade-offs caused by management strategies and to determine net climate mitigation benefits. Changes in soil carbon stocks are only one piece in climate mitigation accounting: GHG emissions (CO2, CH4, N2O) from soils, livestock, manure management systems, transportation, and farm infrastructure need to be considered as well, as do land requirements and implications for water quality. A narrow focus on soil organic carbon in certain locations could potentially cause net negative consequences for climate and the local environment from a broader perspective. Life cycle assessment (LCA) is an important tool in this context, and useful frameworks exist that can be considered in future Vermont-based efforts using local data (Rowntree et al., 2020; Terlouw et al., 2021).
Stakeholders in Vermont are currently exploring Payment for Ecosystem Services (PES) schemes that could potentially include soil carbon. Based on our assessment of the evidence and literature presented in this chapter, we can answer some important questions that need to be addressed in PES design as follows:
- Are some Vermont farms sequestering carbon from the atmosphere into soils?
- YES, this is very likely given the evidence in TNC’s AgEvidence and the number of producers using conservation practices in Vermont.
- Should these farmers be rewarded for their carbon stewardship?
- YES, we think some mechanism is needed to reward and incentivize carbon stewardship in agriculture.
- Can we assume that certain practices will increase permanent C sequestration?
- NO, the existing evidence indicates that results will vary for practices based on site-specific factors, and that any carbon sequestered may be reintroduced to the atmosphere due to future management or climatic factors.
- Is it possible to accurately estimate or model C sequestration without measurements?
- NO, models need to be tested against local data to build confidence in their predictions.
- Should soil carbon sequestration be paid for to offset GHG emissions from other sectors (e.g., fossil fuel use)?
- NO, it would be unwise to invest in offsetting the more certain impact of GHG emissions with the uncertain and reversible C sequestration associated with agricultural soil management.
On the last question, we concur with scholars who suggest that targets and accounting for negative emissions technologies, including agricultural soil carbon sequestration, should be managed separately from existing and future targets for emissions reduction (McLaren et al., 2019). Efforts to balance emissions and offsets within a single accounting scheme to move towards “net-zero” could potentially deter or delay GHG emissions reduction, and therefore negative emissions technologies should be pursued in addition to rapid emissions reduction (McLaren et al., 2019). A recent report covering protocols for soil carbon sequestration concludes that:
“Consistent accounting and verification of direct emission reductions during agricultural production — reduced nitrous oxide emissions via improved nutrient management, reduced carbon dioxide emissions via reduced tractor use and reduced methane emissions from improved manure management — and from avoided land conversion is a less risky and permanent climate solution [as compared to soil carbon sequestration] for supply chain and other public investment.”
(Oldfield et al., 2021)
10.7 Recommendations for Next Steps in Vermont
Agricultural soil carbon sequestration deserves increased attention in Vermont. However, more research is needed to strengthen the scientific evidence for agricultural soil carbon sequestration in the state, which is currently weak. As mentioned previously, this research could include local benchmark sites undergoing long-term experiments, well-characterized chronosequences or paired sampling sites, and testing the abilities of models to adequately replicate local field and lab data (Smith et al., 2020). Without this research, pursuing carbon offsets or payments associated with soil organic carbon storage on working agricultural lands in Vermont could fail to achieve desired outcomes due to the existing uncertainty concerning climate mitigation benefits, and could in some cases even be counterproductive. Verification of changes in soil organic carbon storage will be critical for any PES program and monitoring can include a combination of direct measurements and models that have been well-calibrated to local agroecosystems. The latter will be necessary for inclusion of GHG fluxes and offsite C impacts, which should be considered in concert with onsite soil organic carbon changes to avoid unintended climate and environmental consequences caused by trade-offs. A challenge will be keeping such monitoring programs cost-effective and manageable. Furthermore, it is important to establish consistent protocols for soil organic carbon estimation and quantification of net GHG emissions reductions in Vermont. Variation in protocols employed would make it difficult to ensure net climate benefits have been achieved and the resulting lack of comparability and standardization would be especially problematic in carbon accounting schemes (Oldfield et al., 2021).
There are several critical knowledge gaps and programmatic questions that need to be answered before launching a large-scale program in Vermont focused on soil carbon sequestration on working agricultural lands (Box 10-1). Equally important is continuing and expanding soil management strategies that maintain existing soil carbon, especially considering the relatively high organic matter levels observed in many Vermont soils.
Box 10.1: Recommendations for research in Vermont to inform future soil carbon sequestration efforts on working agricultural lands in the state, derived from Rumpel et al. (2020) and Amelung et al. (2020).
- Assessment of current soil organic carbon stocks and soil degradation status.
- Assessment of yield gaps and reliable predictions of yield changes with soil organic carbon increases.
- Assessment of soil organic carbon changes by practice and the soil organic carbon storage potential using long-term observations, experimental farm plots, existing state soils data, and appropriate chronosequences.
- Estimation of additional nutrient (N and P) requirements for sustainable C sequestration at the level desired.
- Assessment of the vulnerability of soil organic carbon stocks to determine permanence of carbon storage.
- Selection of methods to account for full life-cycle GHG emissions in farming systems and transfers of organic material that may reduce stored carbon elsewhere.
- Broad ensemble of policies and bottom-up approaches including farmer incentives, standards, and actions to scale up adoption of C sequestering practices.
While the existing evidence for consistent soil organic carbon gains for conservation practices reviewed here is weak, there is more substantial and consistent evidence that many practices recommended for sequestering carbon improve soil aggregation and water infiltration and retention (Dabney, 1998; Jones et al., 1994; Palm et al., 2014). For example, reducing tillage is consistently found to improve soil aggregation (Nunes et al., 2020; Karlen et al., 2019; Mann et al., 2019) which contributes to improved water infiltration and reduced surface runoff. Similarly, cover crops can improve soil water infiltration capacity and aggregation (Magdoff & Van Es, 2009). Implementing conservation practices as strategies for adapting to climate change and improving farm resilience can likely offer immediate benefits while also providing opportunities to systematically assess the carbon sequestration potential of practices across a range of soil types and farm business models.
Systematic assessment of carbon sequestration potential on agricultural lands in Vermont will require participatory research conducted in partnership with farmers and land managers. We advocate for designing future research that builds on the questions, experiences, and observations of producers who are implementing carbon-focused management practices. Incorporating the complementary expertise of farmers, scientists, and agricultural service providers will be necessary to parse the complex dynamics involved in soil carbon sequestration specifically and the interactions between agricultural soils and a changing climate more broadly. It is important to note, however, that a participatory, long-term approach to soil research may require shifts in the expectations and limitations imposed by current funding mechanisms.
10.8 Traceable Accounts
Traceable accounts describe the confidence level—the degree of certainty in the scientific evidence—for each key message resulting from this chapter. This analysis is based on the U.S. Global Change Research Program guidance in the Fourth National Climate Assessment (USGCRP, 2018).
Confidence Level
- Very High – Strong evidence (established theory, multiple sources, confident results, well-documented and accepted methods, etc.), high consensus
- High – Moderate evidence (several courses, some consistency, methods vary, and/or documentation limited, etc.), medium consensus
- Medium – Suggestive evidence (a few sources, limited consistency, models incomplete, methods emerging, etc.), competing schools of thought
- Low – Inconclusive evidence (limited sources, extrapolations, inconsistent findings, poor documentation and/or methods not tested, etc.), disagreement or lack of opinions among experts
Key Message 1
The soil carbon sequestration potential of agricultural management practices in Vermont is uncertain and likely mediated by site-specific factors such as soil type, geography, land use history, and weather.
- Finding: Low to Medium Confidence that practices will result in soil carbon sequestration. Medium confidence for no till and low confidence for other practices discussed in the main text.
- References: See main text.
Key Message 2
Assigning carbon offsets or payments for climate mitigation services provided by Vermont agricultural lands based on practice adoption alone currently lacks a strong scientific foundation.
- Finding: Low Confidence that this approach will yield climate benefits.
- References: See main text.
Key Message 3
Whole-system accounting is required to assess potential trade-offs and to determine net climate change mitigation benefits of soil management strategies.
- Finding: Very High Confidence that this approach is required.
- Reference: See text.
10.9 References
Adair, E. C., Barbieri, L., Schiavone, K., & Darby, H. M. (2019). Manure Application Decisions Impact Nitrous Oxide and Carbon Dioxide Emissions during Non‐Growing Season Thaws. Soil Science Society of America Journal, 83(1), 163-172.
Amelung, W., et al. (2020). Towards a global-scale soil climate mitigation strategy. Nature communications, 11(1), 1-10.
Bakelaar, J., Neher, D. A., & Gilker, R. (2016). Minimal soil quality impact by cold season pasture management in Vermont. Canadian Journal of Soil Science, 97(2), 215-225.
Baker, J. M., Ochsner, T. E., Venterea, R. T., & Griffis, T. J. (2007). Tillage and soil carbon sequestration—What do we really know? Agriculture, ecosystems & environment, 118(1-4), 1-5.
Baldock, J. A., & Skjemstad, J. O. (2000). Role of the soil matrix and minerals in protecting natural organic materials against biological attack. Organic geochemistry, 31(7-8), 697-710.
Basile-Doelsch, I., Balesdent, J., & Pellerin, S. (2020). Reviews and syntheses: The mechanisms underlying carbon storage in soil. Biogeosciences, 17(21), 5223-5242.
Briske, D. D., Derner, J. D., Milchunas, D. G., & Tate, K. W. (2011). An evidence-based assessment of prescribed grazing practices. Conservation benefits of rangeland practices: Assessment, recommendations, and knowledge gaps, 21-74.
Byrnes, R. C., Eastburn, D. J., Tate, K. W., & Roche, L. M. (2018). A global meta‐analysis of grazing impacts on soil health indicators. Journal of environmental quality, 47(4), 758-765.
Campbell, J. L., Sessions, J., Smith, D., & Trippe, K. (2018). Potential carbon storage in biochar made from logging residue: Basic principles and Southern Oregon case studies. PloS one, 13(9), e0203475.
Cool Farm Alliance. Cool Farm Tool https://coolfarmtool.org/ retrieved: 6/13/2019
Dabney, S.M. 1998. Cover crop impacts on watershed hydrology. Journal of Soil and Water Conservation. 53 (3) 207-213.
Derner, J. D., Stanley, C. C., & Ellis, C. (2016). Usable science: Soil health. Rangelands, 38(2), 64-67.
Dittmer, K. M., Darby, H. M., Goeschel, T. R., & Adair, E. C. (2020). Benefits and tradeoffs of reduced tillage and manure application methods in a Zea mays silage system. Journal of Environmental Quality 49: 1236-1250.
Drever et al. (2021). Natural climate solutions for Canada. Science Advances 7: eabd6034.
Fitton, N., Datta, A., Cloy, J.M., Rees, R.M., Topp, C.F.E., Bell, M.J., Cardenas, L.M., Williams, J., Smith, K., Thorman, R. and Watson, C.J., 2017. Modelling spatial and inter-annual variations of nitrous oxide emissions from UK cropland and grasslands using DailyDayCent. Agriculture, Ecosystems & Environment, 250, pp.1-11.
Foster, B. (2021, February 26). Vermont farmers are covering good ground for soil health. VTDigger. www.vtdigger.org.
Ge, Y., Thomasson, J. A., & Sui, R. (2011). Remote sensing of soil properties in precision agriculture: A review. Frontiers of Earth Science, 5(3), 229-238.
Godde, C. M., et al. (2020). Soil carbon sequestration in grazing systems: managing expectations. Climatic Change, 1-7.
Guenet, B., et al. (2021). Can N2O emissions offset the benefits from soil organic carbon storage?. Global Change Biology, 27(2), 237-256.
Hassink, J. (1997). The capacity of soils to preserve organic C and N by their association with clay and silt particles. Plant and soil, 191(1), 77-87.
Huang, X., Terrer, C., Dijkstra, F. A., Hungate, B. A., Zhang, W., & van Groenigen, K. J. (2020). New soil carbon sequestration with nitrogen enrichment: a meta-analysis. Plant and Soil, 454(1), 299-310.
Jemison Jr, J. M., Kersbergen, R., Majewski, C., & Brinton, W. (2019). Soil health of recently converted no-till corn fields in Maine. Communications in Soil Science and Plant Analysis, 50(19), 2384-2396.
Jian, J., Du, X., Reiter, M. S., & Stewart, R. D. (2020). A meta-analysis of global cropland soil carbon changes due to cover cropping. Soil Biology and Biochemistry, 143, 107735.
Jones, O. R., Hauser, V. L. & Popham, T. W. No-tillage effects on infiltration and water conservation on dryland. Transactions of ASAE 37, 473–479 (1994).
Karlen, D. L., Veum, K. S., Sudduth, K. A., Obrycki, J. F., & Nunes, M. R. (2019). Soil health assessment: Past accomplishments, current activities, and future opportunities. Soil and Tillage Research, 195, 104365.
Kersbergen, R. 2012. Reducing fuel and fertilizer costs for corn silage in the Northeast with cover crops and no-till. Final report for NLE-09-287. Sustainable Agriculture Research and Extension. https://projects.sare.org/projectreports/lne09-287/.
Konen, M. E., Jacobs, P. M., Burras, C. L., Talaga, B. J., & Mason, J. A. (2002). Equations for predicting soil organic carbon using loss‐on‐ignition for north central US soils. Soil Science Society of America Journal, 66(6), 1878-1881.
Lal, R. (2018). Digging deeper: A holistic perspective of factors affecting soil organic carbon sequestration in agroecosystems. Global Change Biology, 24(8), 3285-3301.
Leslie, S. (2020, December 20). Why Vermont needs a healthy soils act. VTDigger. www.vtdigger.org.
Liang, B. C., & Mackenzie, A. F. (1992). Changes in soil organic carbon and nitrogen after six years of corn production. Soil Science, 153(4), 307-313.
Liebig, M. A., G. Varvel, and W. Honeycutt. (2010). Chapter 1: Guidelines for site description and soil sampling, processing, analysis, and archiving. GRACEnet Protocols. https://www.ars.usda.gov/anrds/gracenet/gracenet-protocols/
Lobsey, C. R., & Viscarra Rossel, R. A. (2016). Sensing of soil bulk density for more accurate carbon accounting. European Journal of Soil Science, 67(4), 504-513.
Luo, Z., Wang, E., & Sun, O. J. (2010). Can no-tillage stimulate carbon sequestration in agricultural soils? A meta-analysis of paired experiments. Agriculture, ecosystems & environment, 139(1-2), 224-231.
Magdoff, F., & Van Es, H. (2009). Building Soils for Better Soil: Sustainable Soil Management, Chapter 4: The Living Soil.
Mann, C., Lynch, D., Fillmore, S., & Mills, A. (2019). Relationships between field management, soil health, and microbial community composition. Applied Soil Ecology, 144, 12-21.
McLaren, D. P., Tyfield, D. P., Willis, R., Szerszynski, B., & Markusson, N. O. (2019). Beyond “net-zero”: a case for separate targets for emissions reduction and negative emissions. Frontiers in Climate, 1, 4.
Moebius-Clune, B. N. (2016). Comprehensive assessment of soil health: The Cornell framework manual. Cornell University.
Mosier, S., Apfelbaum, S., Byck, P., Calderon, F., Teague, R., Thompson, R., & Cotrufo, M. F. (2021). Adaptive multi-paddock grazing enhances soil carbon and nitrogen stocks and stabilization through mineral association in southeastern US grazing lands. Journal of Environmental Management, 288, 112409.
Mulder, V. L., De Bruin, S., Schaepman, M. E., & Mayr, T. R. (2011). The use of remote sensing in soil and terrain mapping—A review. Geoderma, 162(1-2), 1-19.
NREL (National Renewable Energy Lab), Colorado State University and USDA. COMET-farm http://cometfarm.nrel.colostate.edu/ retrieved: 6/13/2019
Neher, Deborah; Horner, Katie; von Wettberg, Eric Bishop; Scarborough, Matt; Harris, Jeanne; Darby, Heather M.; Badireddy, Appala Raju; Roy, Eric D.; Farley, Joshua C.; Faulkner, Joshua; and White, Alissa, “Resilient Soils for Resilient Farms: An Integrative Approach to Assess, Promote and Value Soil Health for Small- and Medium-Size Farms” (2021). USDA Agricultural Research Service (ARS) Center. 7.
Nelson, D.W., and L.E. Sommers. 1996. Total carbon, organic carbon, and organic matter. p. 961-1010. In: Sparks, D.L. (ed.) Methods of soil analysis. Part 3. Chemical methods. SSSA Book Series No. 5. SSSA and ASA, Madison, WI.
Newton, P., Civita, N., Frankel-Goldwater, L., Bartel, K., & Johns, C. (2020). What Is Regenerative Agriculture? A Review of Scholar and Practitioner Definitions Based on Processes and Outcomes. Frontiers in Sustainable Food Systems, 4, 194.
Nordborg M (2016) Holistic management – a critical review of Allan Savory’s grazing method. SLU/EPOK – Centre for Organic Food & Farming & Chalmers, Uppsala.
Nunes, M. R., Karlen, D. L., Veum, K. S., Moorman, T. B., & Cambardella, C. A. (2020). Biological soil health indicators respond to tillage intensity: A US meta-analysis. Geoderma, 369, 114335.
Ogle, S.M., et al. (2019). Ch. 5 – Cropland. In: 2019 Refinement to the IPCC Guidelines for National Greenhouse Gas Inventories. https://www.ipcc-nggip.iges.or.jp/public/2019rf/vol4.html
Oldfield, E.E., A.J. Eagle, R.L Rubin, J. Rudek, J. Sanderman, D.R. Gordon. 2021. Agricultural soil carbon credits: Making sense of protocols for carbon sequestration and net greenhouse gas removals. Environmental Defense Fund, New York, New York. edf.org/sites/default/files/content/agricultural-soil-carbon-credits-protocolsynthesis.pdf.
Olson, K. R., & Al-Kaisi, M. M. (2015). The importance of soil sampling depth for accurate account of soil organic carbon sequestration, storage, retention and loss. Catena, 125, 33-37.
Owen, J. J., Parton, W. J., & Silver, W. L. (2015). Long‐term impacts of manure amendments on carbon and greenhouse gas dynamics of rangelands. Global change biology, 21(12), 4533-4547.
Palm, S., Blanco-Canqui, H., DeClerck, F., Gatere, L. & Grace, P. Conservation agriculture and ecosystem services: an overview. Agriculture, Ecosystems and Environment 187, 87–105 (2014).
Paustian, K., Lehmann, J., Ogle, S., Reay, D., Robertson, G. P., & Smith, P. (2016). Climate-smart soils. Nature, 532(7597), 49-57.
Paustian, K., Larson, E., Kent, J., Marx, E., & Swan, A. (2019). Soil C sequestration as a biological negative emission strategy. Frontiers in Climate, 1, 8.
Paustian, K., C. Chenu, R. Conant, F. Cotrufo, R. Lal, P. Smith, J.F. Soussana. (2020). Climate mitigation potential of regenerative agriculture is significant! https://static1.squarespace.com/static/5c3780907c9327dc2a2e8c64/t/5ee0ed849bd3430a15121b12/1591799173373/Regen+Ag+pdf+061020.pdf
Poeplau, C., & Don, A. (2015). Carbon sequestration in agricultural soils via cultivation of cover crops–A meta-analysis. Agriculture, Ecosystems & Environment, 200, 33-41.
Poulton, P., Johnston, J., Macdonald, A., White, R., & Powlson, D. (2018). Major limitations to achieving “4 per 1000” increases in soil organic carbon stock in temperate regions: Evidence from long‐term experiments at Rothamsted Research, United Kingdom. Global Change Biology, 24(6), 2563-2584.
Powlson, D. S., Stirling, C. M., Jat, M. L., Gerard, B. G., Palm, C. A., Sanchez, P. A., & Cassman, K. G. (2014). Limited potential of no-till agriculture for climate change mitigation. Nature Climate Change, 4(8), 678-683.
Powlson, D., R. Boddey, K. Cassman, P. Chivenge, K. Giller, K. Goulding, C. van Kessel, C. Palm, and J.W. van Groenigen. (2020). Letter to World Resources Institute Regarding Soil Carbon Sequestration. https://scholar.princeton.edu/sites/default/files/tsearchi/files/letter_from_powlson_et_al._to_wri_in_support_of_soil_carbon_chapter_august_2020_.pdf
Ranganathan, J., Waite, R., Searchinger, T., & Zionts, J. (2020). Regenerative Agriculture: Good for Soil Health, but Limited Potential to Mitigate Climate Change.
Roche, L. M., Cutts, B. B., Derner, J. D., Lubell, M. N., & Tate, K. W. (2015). On-ranch grazing strategies: context for the rotational grazing dilemma. Rangeland Ecology & Management, 68(3), 248-256.
Rowntree, J. E., Stanley, P. L., Maciel, I. C., Thorbecke, M., Rosenzweig, S. T., Hancock, D. W., Guzman, A., & Raven, M. R. (2020). Ecosystem Impacts and Productive Capacity of a Multi-Species Pastured Livestock System. Frontiers in Sustainable Food Systems, 4, 232.
Rumpel, C., et al. (2020). The 4p1000 initiative: Opportunities, limitations and challenges for implementing soil organic carbon sequestration as a sustainable development strategy. Ambio, 49(1), 350-360.
Shackley, S., Hammond, J., Gaunt, J., & Ibarrola, R. (2011). The feasibility and costs of biochar deployment in the UK. Carbon Management, 2(3), 335-356.
Schlesinger, W. H. (2000). Carbon sequestration in soils: some cautions amidst optimism. Agriculture, Ecosystems & Environment, 82(1-3), 121-127.
Schlesinger, W. H., & Bernhardt, E. S. (2013). Biogeochemistry: An Analysis of Global Change. Academic Press.
Schulz, K., Voigt, K., Beusch, C., Almeida-Cortez, J. S., Kowarik, I., Walz, A., & Cierjacks, A. (2016). Grazing deteriorates the soil carbon stocks of Caatinga forest ecosystems in Brazil. Forest Ecology and Management, 367, 62-70.
Searchinger, T. and J. Ranganathan. (2020). INSIDER: Further Explanation on the Potential Contribution of Soil Carbon Sequestration on Working Agricultural Lands to Climate Change Mitigation. World Resources Institute. https://www.wri.org/insights/insider-further-explanation-potential-contribution-soil-carbon-sequestration-working
E Slessarev, J Zelikova, J Hamman, D Cullenward, J Freeman (2021) “Depth matters for soil carbon accounting” CarbonPlan. https://carbonplan.org/research/soil-depth-sampling
Smith, P. (2016). Soil carbon sequestration and biochar as negative emission technologies. Global change biology, 22(3), 1315-1324.
Smith, P. (2004). How long before a change in soil organic carbon can be detected?. Global Change Biology, 10(11), 1878-1883.
Smith, P., et al. (2020). How to measure, report and verify soil carbon change to realize the potential of soil carbon sequestration for atmospheric greenhouse gas removal. Global Change Biology, 26(1), 219-241.
Spohn, M. (2020). Increasing the organic carbon stocks in mineral soils sequesters large amounts of phosphorus. Global change biology, 26(8), 4169-4177.
Stanley, P. L., Rowntree, J. E., Beede, D. K., DeLonge, M. S., & Hamm, M. W. (2018). Impacts of soil carbon sequestration on life cycle greenhouse gas emissions in Midwestern USA beef finishing systems. Agricultural Systems, 162, 249-258.
Stewart, C. E., Paustian, K., Conant, R. T., Plante, A. F., & Six, J. (2007). Soil carbon saturation: concept, evidence and evaluation. Biogeochemistry, 86(1), 19-31.
Teague, R., Provenza, F., Kreuter, U., Steffens, T., & Barnes, M. (2013). Multi-paddock grazing on rangelands: why the perceptual dichotomy between research results and rancher experience?. Journal of Environmental management, 128, 699-717.
Terlouw, T., Bauer, C., Rosa, L., & Mazzotti, M. (2021). Life cycle assessment of carbon dioxide removal technologies: A critical review. Energy & Environmental Science, 14(4), 1701-1721.
USGCRP. (2018). Impacts, Risks, and Adaptation in the United States: Fourth National Climate Assessment, Volume II: [Reidmiller, D.R., C.W. Avery, D.R. Easterling, K.E. Kunkel, K.L.M. Lewis, T.K. Maycock, and B.C. Stewart (eds.)]. U.S. Global Change Research Program, Washington, DC, USA, 1515 pp. doi: 10.7930/NCA4.2018.
van Groenigen, J. W., van Kessel, C., Hungate, B. A., Oenema, O., Powlson, D. S., & van Groenigen, K. J. (2017). Sequestering Soil Organic Carbon: A Nitrogen Dilemma. Environmental science & technology, 51(9), 4738.
White Oaks Pastures. (2019). Study: White Oak Pastures Beef Reduces Atmospheric Carbon. https://blog.whiteoakpastures.com/blog/carbon-negative-grassfed-beef
Wijewardane, N. K., Ge, Y., Wills, S., & Libohova, Z. (2018). Predicting physical and chemical properties of US soils with a mid‐infrared reflectance spectral library. Soil Science Society of America Journal, 82(3), 722-731.
Woolf, D., Amonette, J. E., Street-Perrott, F. A., Lehmann, J., & Joseph, S. (2010). Sustainable biochar to mitigate global climate change. Nature communications, 1(1), 1-9.